- Department of Plant Pathology and Weed Research, Agricultural Research Organization, Volcani Institute, Rishon LeZion, Israel
Botrytis cinerea is the causative agent of gray mold disease, and infects more than 1400 plant species, including important crop plants. In tomato, B. cinerea causes severe damage in greenhouses and post-harvest storage and transport. Plant viruses of the Tobamovirus genus cause significant damage to various crop species. In recent years, the tobamovirus tomato brown rugose fruit virus (ToBRFV) has significantly affected the global tomato industry. Most studies of plant-microbe interactions focus on the interaction between the plant host and a single pathogen, however, in agricultural or natural environments, plants are routinely exposed to multiple pathogens. Here, we examined how preceding tobamovirus infection affects the response of tomato to subsequent infection by B. cinerea. We found that infection with the tobamoviruses tomato mosaic virus (ToMV) or ToBRFV resulted in increased susceptibility to B. cinerea. Analysis of the immune response of tobamovirus-infected plants revealed hyper-accumulation of endogenous salicylic acid (SA), upregulation of SA-responsive transcripts, and activation of SA-mediated immunity. Deficiency in SA biosynthesis decreased tobamovirus-mediated susceptibility to B. cinerea, while exogenous application of SA enhanced B. cinerea symptoms. These results suggest that tobamovirus-mediated accumulation of SA increases the plants’ susceptibility to B. cinerea, and provide evidence for a new risk caused by tobamovirus infection in agriculture.
Introduction
Throughout their life cycle, plants are continuously challenged by various types of pathogens. To cope with these challenges, plants evolved a two-tiered immune system that enables them to mitigate the effects of invading pathogens (Jones and Dangl, 2006; Delplace et al., 2020). Following pathogen infection, plants can develop induced resistance to cope with future attacks. Induced resistance is generally divided into systemic acquired resistance (SAR) and induced systemic resistance (ISR) (Walters and Heil, 2007). SAR, commonly triggered by local infection, can provide long-term resistance to subsequent infection (Klessig et al., 2018). SAR generally relies on salicylic acid (SA), which has been shown to increase in plant tissues during pathogenesis (Yalpani et al., 1991).
Countless studies in the past few decades have focused on elucidating the interactions between various plant hosts and the pathogens that threaten them, however, most of these studies investigate the interaction between the plant host and a single pathogen, in controlled conditions. In agricultural or natural environments, plants are routinely exposed to more than one pathogen at a given time. Such infections can be challenging to mimic in research settings. Some studies have attempted to elucidate the nature of particular diseases caused by a combination of pathogens (Lamichhane and Venturi, 2015; Fang et al., 2021; Tan et al., 2021). In some cases, one pathogen can prime immunity against another (Orton and Brown, 2016), while in other cases, a prior or simultaneous pathogen infection will increase plant susceptibility towards additional pathogens (Spoel et al., 2007; Cooper et al., 2008; Zhang et al., 2022b).
Plant viruses of the genus Tobamovirus (family Virgaviridae) are some of the most economically important plant pathogens. Tobamoviruses are highly infectious, and cause significant damage to various crop species. They are transmitted by mechanical contact: through e.g., workers’ hands, agricultural tools and soil (Broadbent, 1976), and the diseases symptoms caused by these viruses include stunting, leaf blistering, malformations and yellow mosaic patterns, fruit spotting and marbling and suppression of root branching (Luria et al., 2017; Vaisman et al., 2022). The tomato mosaic virus (ToMV) was isolated in the early 20th century as a virus that severely impacts tomato crops (Broadbent, 1976). Since then, effective ToMV-resistance genes have been discovered in tomato, and currently most modern cultivars are immune to this virus (Hall, 1980). In recent years, the tobamovirus tomato brown rugose fruit virus (ToBRFV) (Zhang et al., 2022a) has caused significant damage to tomato crops, leading to reductions in fruit quality and yield in various parts of the world. This is largely due to the ability of the ToBRFV movement protein to overcome the durable tomato resistance gene, Tm-22 (Luria et al., 2017; Hak and Spiegelman, 2021). Since there is currently no available commercial genetic resistance to ToBRFV, the only means to control ToBRFV is by effective detection, containment and disinfection (Oladokun et al., 2019; Panno et al., 2019; Davino et al., 2020; Alon et al., 2021; Samarah et al., 2021; Dombrovsky et al., 2022).
In many cases, viral infection has been reported to exacerbate the symptoms of another pathogen infection, leading to more severe disease (Bains and Parkash, 1984; Omar et al., 1986; Meyer and Pataky, 2010; Tollenaere et al., 2017; Philosoph et al., 2018). For example, the tobamovirus cucumber green mottle mosaic virus (CGMMV) has been shown to increase the severity of Pythium wilting in cucumber under various environmental conditions (Philosoph et al., 2018; Philosoph et al., 2019). Other outcomes of co-infection with viruses and phytopathogens have been reported, with viral infection reducing disease susceptibility in certain cases (Latch and Potter, 1977; Varughese and Griffiths, 1983). However the molecular pathways determining these interactions remain unclear.
Botrytis cinerea (B. cinerea, Bc) is the causative agent of gray mold disease (Williamson et al., 2007). B. cinerea infects upwards of 1400 plant hosts, many of them crop plants, and causes severe damage in greenhouses and post-harvest storage and transport (Williamson et al., 2007; Elad et al., 2016; Fillinger and Elad, 2016). Xanthomonas euvesicatora (Xcv) causes bacterial leaf spot (BLS) on peppers and tomatoes. BLS severely reduces plant productivity and yield (Jones et al., 2004). Since B. cinerea and X. euvesicatoria are important pathogens in tomato agricultural cropping (Moss et al., 2007; Fillinger and Elad, 2016), and since the tobamovirus ToBRFV has become widespread in worldwide tomato agriculture (Zhang et al., 2022a), we were interested to examine the impact of the interaction between B. cinerea/X. euvesicatoria and tobamoviruses on tomato disease response.
Different pathogens can activate common or different pathways in their plant host. The nature of the defense pathways activated during infection with more than one pathogen will likely influence the eventual resistance or susceptibility to each pathogen. B. cinerea, primarily a necrotrophic plant pathogen, is known to activate Jasmonic acid (JA) pathway defenses (Rowe et al., 2010; Mehari et al., 2015; Fillinger and Elad, 2016). Mutant plants with impaired JA content or sensing are notoriously sensitive to B. cinerea infection (AbuQamar et al., 2008; Gupta et al., 2021a; Gupta et al., 2022). B. cinerea was also demonstrated to activate SA-dependent pathways in planta, promoting pathogenesis (El Oirdi et al., 2011; Rossi et al., 2011). Tobamoviruses are known to activate a different plant defense response pathway, the salicylic acid (SA) pathway (White, 1979; Gaffney et al., 1993; Murphy and Carr, 2002). While JA confers defense against necrotrophic pathogens, SA is largely thought to confer protection against biotrophic pathogens (Yang et al., 2015). The competitive crosstalk between the JA and SA pathways determines the precise defense response to the specific attacker (Thaler et al., 2012; Caarls et al., 2015; Aerts et al., 2021). In this work, we found that infection with the tobamoviruses ToMV or ToBRFV resulted in increased B. cinerea incited disease, likely through increases in endogenous SA and activation of the SA pathway. Our results suggest that manipulation of plant defense pathways by viruses may lead to unexpected outcomes, which include increased susceptibility to additional pathogens. Given that the increased pathogen susceptibility may ultimately cause more damage than the original virus, future management strategies for plant viruses should take into account both the primary damage caused by viruses, and the secondary effect of plant viruses on susceptibility to additional pathogens such as B. cinerea.
Materials and methods
Plant materials and growth conditions
Solanum lycopersicum cv Moneymaker (“Mm”) and the transgenic SA degrading NahG line (Gupta et al., 2021b) were grown from seeds in soil (Green Mix 443; Even‐Ari, Ashdod, Israel) in a growth chamber, under long day conditions (16 hr:8 hr, light:dark) at 24°C.
Tobamovirus infection
Tobamovirus infection was performed as described (Vaisman et al., 2022). Briefly, 5 gr of symptomatic leaves from plants infected with either ToMV (accession no. NC_002692.1) or ToBRFV (accession no. KX619418.1) were crushed using mortar and pestle and supplemented with carborundum powder and 50 ml of phosphate buffer (0.01 M NaH2PO4) 0.01 M. Inoculation was done on cotyledons of two-week-old tomato seedlings by gently rubbing them with the crushed leaf solution.
B. cinerea inoculation using mycelial plugs
B. cinerea (isolate Bc16) was cultured on potato dextrose agar (PDA) (Difco Lab) and incubated at 22°C for 3-5 days. Leaves number 5-6 from 5-week-old tomato plants (three weeks after tobamovirus inoculation) were excised and placed in humid chambers. Mycelial plugs (5 mm diameter) from a 3-day-old culture were taken using a cork-borer from colony margins, and placed mycelial side down on the adaxial surface of each leaflet. Inoculated leaves were kept in a humid growth chamber at 21°C for three days. Assay was repeated four independent times.
Xanthomonas euvesicatoria inoculation by infiltration
X. euvesicatoria bacterial cultures were grown in LB medium containing 100 mg/L of rifampicin and 300 mg/L of streptomycin, overnight at 28°C (O’Donnell et al., 2001). Log phase bacterial cultures were harvested and re-suspended in 10 mM MgCl2, at a final concentration of 104 CFU/mL (OD600 = 0.0002). The fourth leaf of 5-week-old tomato plants (3 weeks after tobamovirus inoculation) was infiltrated with the bacterial suspensions using a needless syringe. To determine CFU, five days after injection, three leaf discs of 0.9 cm diameter were sampled from the second left-hand lateral leaflet from the inoculated leaf of at least five plants, and ground in 1 mL of 10 mM MgCl2. CFU were determined by plating serial dilutions, and counting the resultant colonies (Lund et al., 1998). 10 mM MgCl2 without pathogen inoculation was used as a negative control. Water-soaked lesions were measured 10 days post inoculation (dpi) as previously described (Teper et al., 2018). Assay was repeated three independent times.
Immunity assays
All immunity assays were conducted on mature leaves from ToMV- and ToBRFV- inoculated plants as well as non-inoculated control plants.
ROS measurement was performed as previously described (Leibman-Markus et al., 2017). Leaf disks 0.5 cm in diameter were taken from leaves 5-6 of 4-5-week-old plants. Disks were floated in a white 96-well plate (SPL Life Sciences, Korea) containing 250 μL distilled water for 4–6 h at room temperature. After incubation, water was removed and a ROS measurement reaction containing either 1 μg/mL EIX (Anand et al., 2021), 1 μM flg-22 (PhytoTech labs), or water (mock) was added. Light emission was immediately measured using a luminometer (GloMax® Discover, Promega, USA).
Conductivity assays were performed according to Leibman-Markus et al. (2017). 0.9 cm diameter leaf discs were harvested from leaves 5-6 of 4-5-week-old plants and washed with distilled water for 3 hours in a 50 mL tube. For each sample, five discs were placed in a 10-flask with 1 ml of distilled water, for 48 hours with agitation. After incubation, 1.5 mL of distilled water were added to each sample, and conductivity was measured using a conductivity meter (AZ® Multiparameter pH/Mv/Cond./Temp Meter 86505, Taiwan).
SA treatments
To simulate virus-induced SA production, SA solutions of 1, 2 or 5 mM in distilled water were sprayed to drip onto aerial plant parts. Plants were given four treatments every 3-4 days over a 2-week period, after which disease susceptibility was assessed.
Gene expression and RT-qPCR
For RT-qPCR analyses, total RNA was prepared using Tri-reagent (Sigma-Aldrich) according to the manufacturer’s instructions. Three μg of RNA were converted to first-strand cDNA using reverse transcriptase (Promega, Madison, WI) and oligo-d(T) primers. RT-qPCR was performed according to the Power SYBR Green Master Mix protocol (Life Technologies, Rockville, MD), using a StepOnePlus machine (Thermo Fisher, Waltham, MA, United States). Supplementary Table S1 lists the primers used in this study and primer pair efficiencies. Systemic viral infection was confirmed by quantifying the expression of ToBRFV and ToMV coat protein genes relative to the housekeeping gene TIP41 (Hak and Spiegelman, 2021; Kravchik et al., 2022). Gene expression was normalized to the geometric mean of the expression of two housekeeping genes: Cyclophilin (CYP) (Solyc01g111170), a housekeeping gene previously used in qPCR experiments in tomato (Mascia et al., 2010), and the ribosomal protein L8 (RPL8) (Solyc10g006580), which was previously used for the normalization of gene expression in pathogen assays in tomato (Meller Harel et al., 2014; Gupta et al., 2021a). Relative expression quantification was done using the copy number method for gene expression (D’haene et al., 2010).
SA measurements in tomato leaf tissues
Tissue for SA content quantification was harvested 2 weeks after tobamovirus infection. Each sample consisted of 5 independent biological replicates. SA was measured as described (Shaya et al., 2019; Gupta et al., 2020). Tissue was harvested, frozen, and immediately ground to a fine powder. One gram of powder was suspended in two vials containing 1 mL extraction solvent (ES) (79% isopropanol: 20% methanol: 1% acetic acid) with the addition of 20 ng of labeled internal standard (Sigma). The samples were incubated at 4°C for 1 h with rapid shaking and subsequently centrifuged at 14,000g for 15 min. The supernatant was collected and 500 µL of ES was added to the pellet, with the extraction steps being repeated an additional two times. Combined extracts were then evaporated using speed-vac at room temperature. The dried samples were dissolved in 200 μl of 50% methanol, and filtered through a 0.22 μm cellulose syringe filter. 5–10 μl of each methanol-dissolved sample was injected for each analysis. LC–MS-MS analyses were conducted using a UPLC-TripleQuadrupoleMS (WatersXevo TQMS). Quantification was performed using isotope-labeled internal standards.
Statistical analyses
Data sets were analyzed for normality using the Shapiro-Wilk test. For normally distributed samples, differences between two groups were analyzed for statistical significance using a two tailed t-test, with Welch’s correction for samples with unequal variances, where appropriate. Differences among three groups or more were analyzed for statistical significance using one-way analysis of variance (ANOVA), or Welch’s ANOVA (when samples within the group were determined to have unequal variances). When a significant result for a group in an ANOVA was returned, significance in differences between the means of different samples in the group were assessed using Tukey’s post-hoc test (equal variances), or Dunnett’s post-hoc test (unequal variances). For samples not normally distributed, differences between two groups were analyzed for statistical significance using Mann-Whitney’s U test, and differences among three groups or more were analyzed for statistical significance using Kruskal-Wallis ANOVA, with Dunn’s post-hoc test. All statistical analyses were conducted using Prism9™. All experiments were conducted in at least three biologically independent repeats. The number of replicates and the statistical tests used in each case are indicated for each experiment in the corresponding figure legend.
Results
Infection with tobamoviruses influences susceptibility to additional pathogens
Increased disease susceptibility following virus infection has been reported in several plant hosts (Latch and Potter, 1977; Bains and Parkash, 1984; Omar et al., 1986; Meyer and Pataky, 2010; Philosoph et al., 2018). Although this phenomenon has been known for many years, the molecular mechanism underlying it is still unclear. To explore the effect of tobamoviruses on disease responses in tomato, we used the necrotrophic fungal pathogen Botrytis cinerea, and the hemibiotrophic bacterial pathogen Xanthomonas euvesicatoria. The experimental procedure is depicted in Figure 1. Two-week-old Solanum lycopersicum cv Moneymaker (Mm) plants were inoculated with either ToMV or ToBRFV. Plants were grown for 3 weeks after inoculation, and systemic infection was confirmed by the appearance of systemic viral symptoms and by RT-qPCR on systemic leaf tissue (Figure 1, Supplementary Figure S1). Leaflets of mature leaves were then challenged with either B. cinerea or X. euvesicatoria, and the response to each pathogen was measured by the lesion size at 3 (B. cinerea) or 10 (X. euvesicatoria) days post inoculation (dpi). Infection with ToMV and ToBRFV increased the lesion generated by B. cinerea (Figures 2A, B). The increased disease severity was greater with ToMV as compared to ToBRFV (Figure 2A, B). Infection with ToMV reduced the disease symptoms caused by X. euvesicatoria (Figures 2B, C), though CFU numbers were unaffected (Figure 2D). These results suggest that while ToMV infection did not have an effect on X. euvesicatoria growth, it did affect the host response to this pathogen. Susceptibility to X. euvesicatoria was not significantly affected by ToBRFV infection (Figures 2C, D).
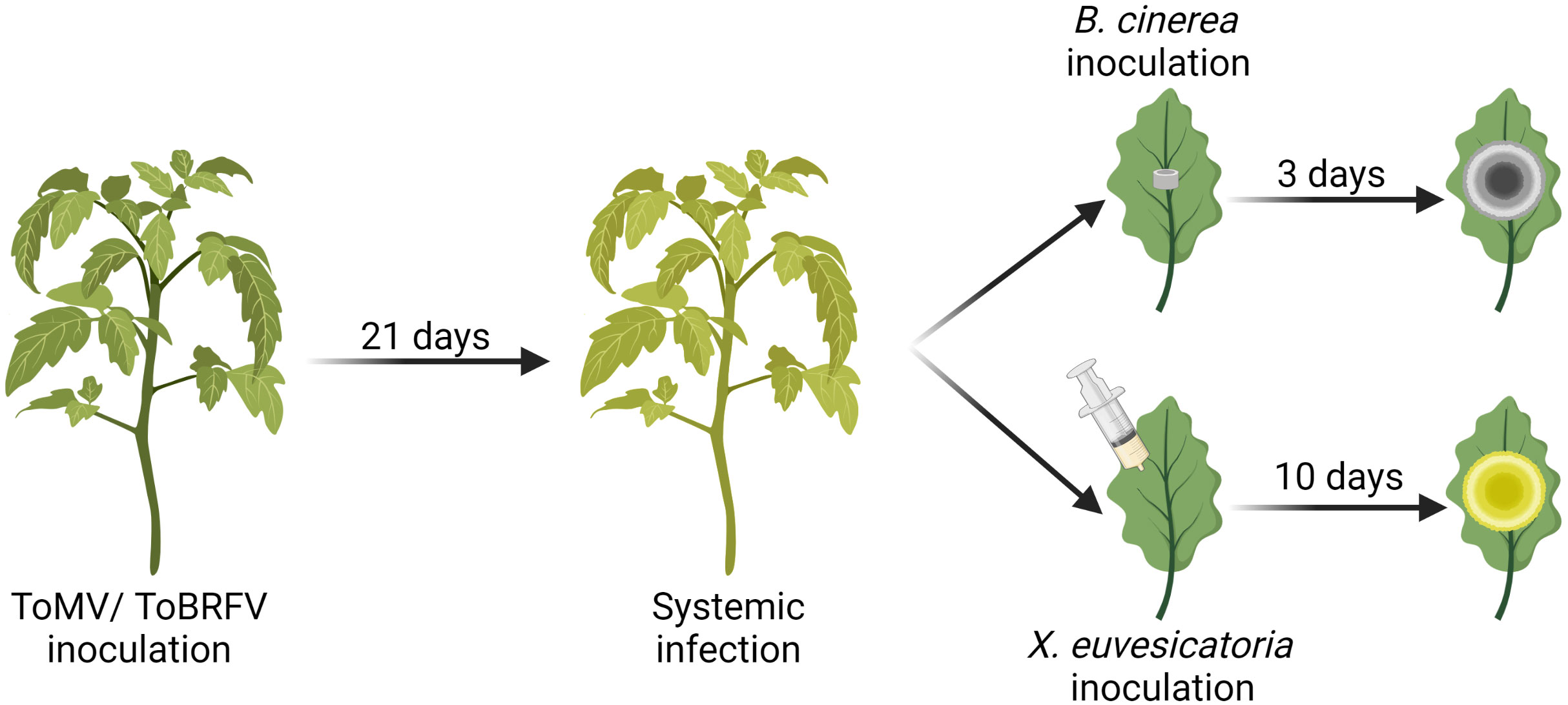
Figure 1 Schematic illustration of the double infection assays performed in this study. Solanum lycopersicum cv. Moneymaker plants were inoculated with ToMV or ToBRFV at 2 weeks of age. Three weeks after inoculation, tobamovirus infection was confirmed by qRT-PCR and by the appearance of visual viral symptoms. At this point, fully expanded mature leaves (number 4-6) were challenged with B. cinerea mycelia plugs or infiltrated with X. euvesicatoria culture (OD600 = 0.0002). Symptoms of B. cinerea and X. euvesicatoria were documented 3 and 10 days after inoculation, respectively. Illustration created using Biorender.com.
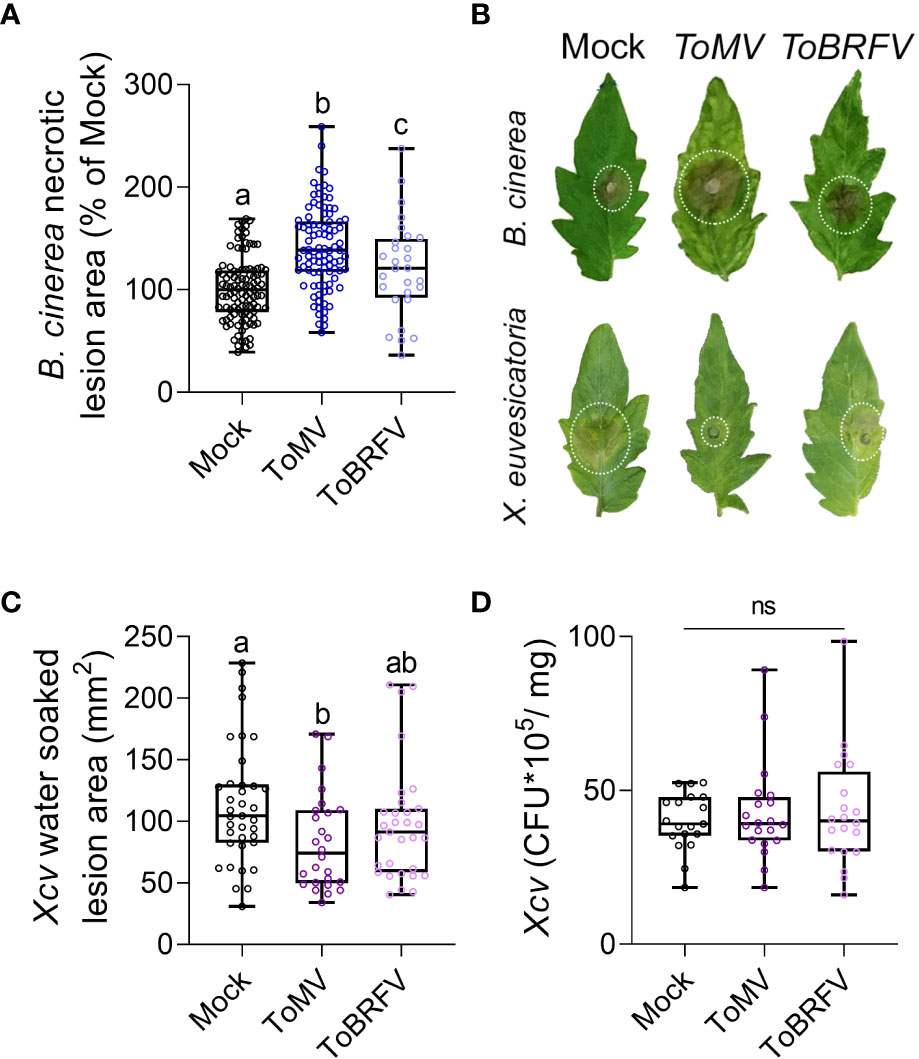
Figure 2 Tobamovirus infection modulates pathogen resistance. S. lycopersicum cv. Moneymaker plants were infected with ToMV or ToBRFV at 2 weeks of age. 3 weeks after viral inoculation, plants were challenged with (B) cinerea 3-day-old mycelia (A, B) or X. euvesicatoria (Xcv, OD600 = 0.0002, B-D) on leaves 4-5. (A) Necrotic lesion area was quantified 3 days after B. cinerea inoculation. Experiment was repeated 4 independent times. (B) Representative images of mock, ToMV- and ToBRFV- infected plants inoculated with B. cinerea or X. euvesicatoria. (C) Water-soaked lesion area was quantified 5 days after X. euvesicatoria inoculation. Experiment was repeated 3 independent times. (D) Leaf tissue samples from 5 individual plants per experiment was ground in MgCl2 and CFU were counted from plated serial dilutions 5 days after X. euvesicatoria inoculation. Experiment was repeated 3 independent times. Boxplots represent inner quartile ranges (box), outer quartile ranges (whiskers), median (line in box), all points shown. Different letters indicate statistically significant differences between samples in one way ANOVA with Tukey’s post hoc test (A, D), or in Welch’s ANOVA with Dunnett’s post hoc test (C). A: N>30, p<0.038. C: N>30, p<0.018. D: N=20. ns, not significant.
Infection with tobamoviruses affects cellular immunity and defense gene expression
To examine the underlying factors modulating disease susceptibility following virus infection, we next assayed immune system activation and defense gene expression following infection with ToMV and ToBRFV. Both viruses increased ROS production in response to elicitation with the bacterial flagellin peptide flg-22 (Pizarro et al., 2020) (Figures 3A, B), while pre-infection by ToMV also promoted ROS production in response to the fungal elicitor EIX (ethylene-inducing xylanase (Anand et al., 2021), Figures 3D, E). ToMV caused an increase in basal conductivity (Figure 3C), and both viruses increased conductivity in response to EIX treatment (Figure 3F). These results suggest that tobamovirus infection alters the plant immune response to pathogen elicitors.
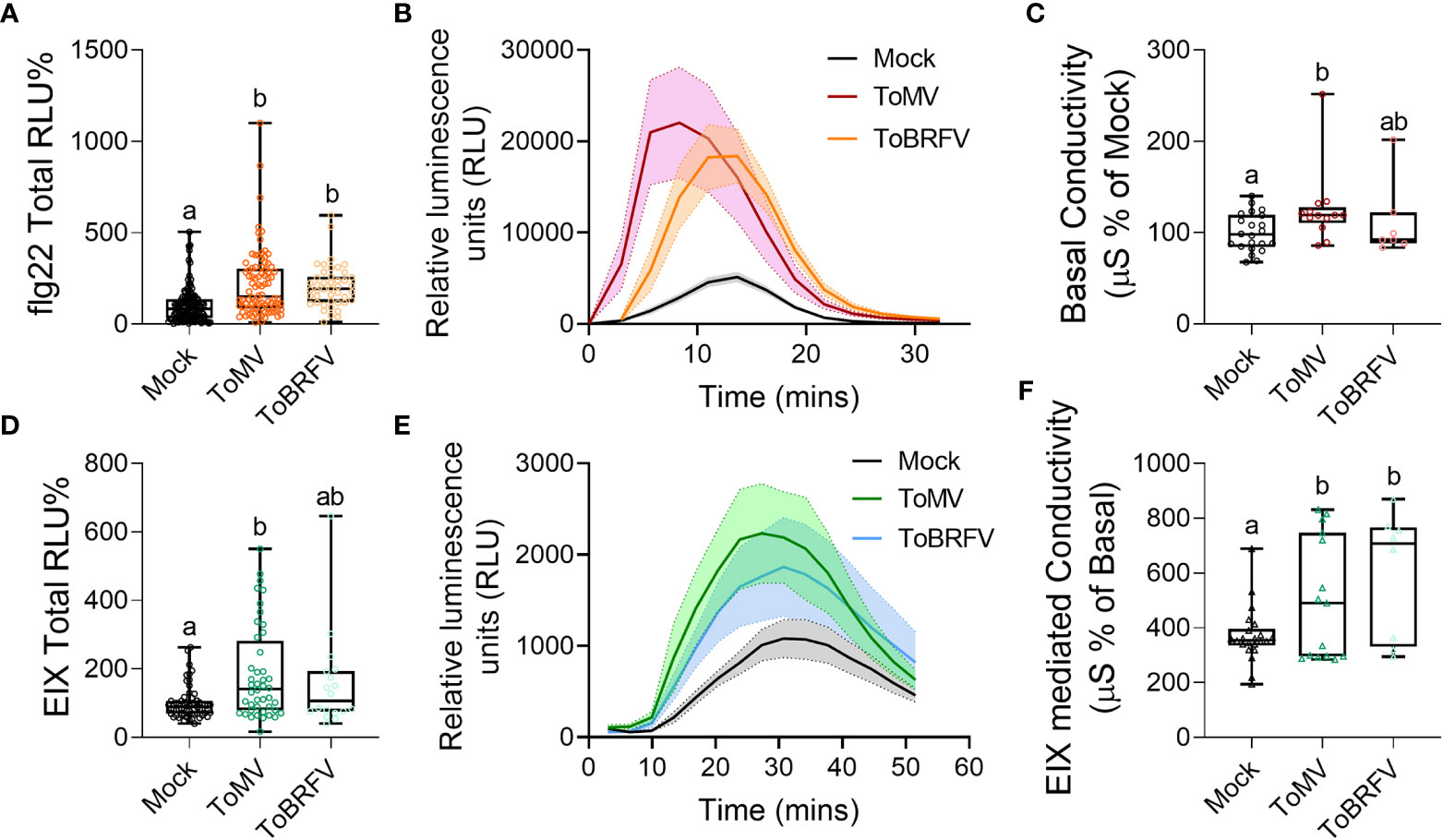
Figure 3 Tobamovirus infection modulates plant immunity. S. lycopersicum cv. Moneymaker plants were infected with ToMV or ToBRFV at 2 weeks of age. Three weeks after viral inoculation, plants were challenged with the immunity elicitors flg22 (A, B) or EIX (D-F), or wounded (C). Reactive oxygen species (ROS) burst in response to the bacterial elicitor flg-22 (A, B) or the fungal elicitor EIX (D, E), and electrolyte leakage in response to wounding (C) or EIX (F) were quantified. (A, D): Total ROS produced, expressed as Relative luminescence units (RLU), in % of Mock. (B, E): Kinetics of the ROS burst. Line indicates mean of each time point, shaded area depicts SE. (C): Basal conductivity as a result of wounding. F: EIX-mediated conductivity. At least three independent experiments were conducted in all cases, (A, B): N≥45, (D, E): N≥18, (C): N≥7, (F): N≥8. (A, C, D, F): Boxplots represent inner quartile ranges (box), outer quartile ranges (whiskers), median (line in box), all points shown. Different letters indicate statistically significant differences between samples in Welch’s ANOVA with Dunnett’s post hoc test (A, C, D), or in one way ANOVA with Tukey’s post hoc test (F). A: p<0.0001; C: p<0.023; D: p<0.0002; F: p<0.046.
To gain insights into the immune pathways altered during viral infection, defense gene transcripts were quantified using RT-qPCR (Figure 4). The SA pathway genes pathogenesis related 1a (PR1a), PR1b, and pathogen-inducible 5 (Pti-5) (Yalpani et al., 1991; Gu et al., 2002), were up-regulated by both ToMV and ToBRFV-infected plants (Figure 4A). In marked contrast, the SA receptor nonexpressor of pathogenesis-related genes 1 (NPR1) and the SA biosynthetic pathway gene phenylalanine lyase (PAL) (Cao et al., 1997; Lin et al., 2004; Kim and Hwang, 2014) were down-regulated following tobamovirus infection, possibly due to a negative feedback mechanism curbing the SA response (Figure 4A). In addition, the JA pathway genes lipoxygenase D (LoxD), ethylene response factor 1 (ERF1), and jasmonate zim domain protein (JAZ) (Ishiga et al., 2013; Meller Harel et al., 2014; Gupta et al., 2022) were all repressed by infection with tobamoviruses (Figure 4B). These results suggest that tobamovirus infection upregulates the SA response pathway while suppressing the JA response pathway.
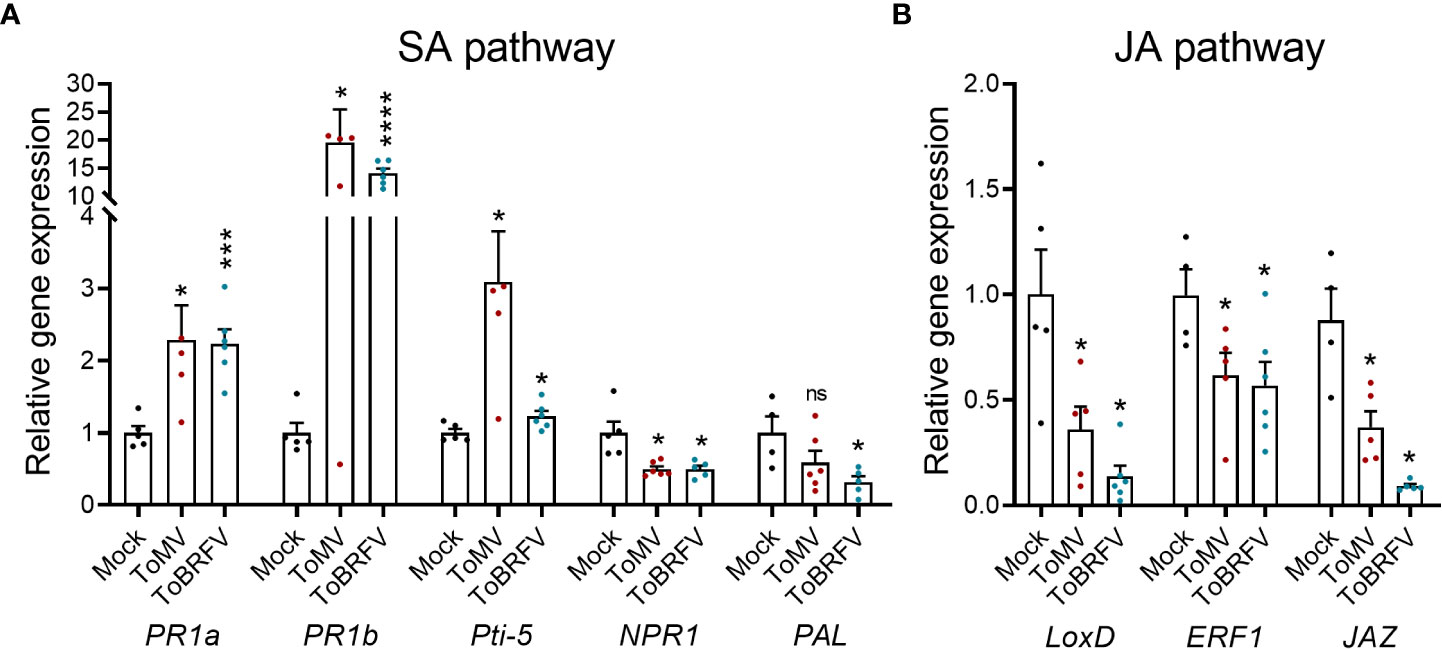
Figure 4 Tobamovirus infection alters SA and JA pathway gene expression. S. lycopersicum cv. Moneymaker plants were infected with ToMV or ToBRFV at 2 weeks of age. Three weeks after viral inoculation, cDNA was prepared from RNA extracted from the fourth leaf of 3 individual plants. SA (A) and JA (B) pathway gene expression was assayed by RT-qPCR. Experiment was repeated twice, N=6. Bars represent mean ± SE, all points shown. Asterisks indicate statistically significant differences in gene expression in virus-infected plants as compared with mock plants, in Welch’s t-rom comparing each gene. *p<0.05, ***p<0.001, ****p<0.0001, ns, not significant.
Alterations in disease susceptibility following tobamovirus infection could result from increases in SA levels
Tobamovirus infection is known to activate SA production in plant cells (Malamy et al., 1990; Conti et al., 2017). Increased SA biosynthesis may therefore explain the activation of SA response pathways due to tobamovirus infection. We directly compared the levels of SA elicited in tomato leaves following infection with ToMV and ToBRFV. While infection with both of these viruses enhanced SA production, ToMV increased leaf SA levels about 25-fold, while ToBRFV increased leaf SA levels about 4-fold (Figure 5A).
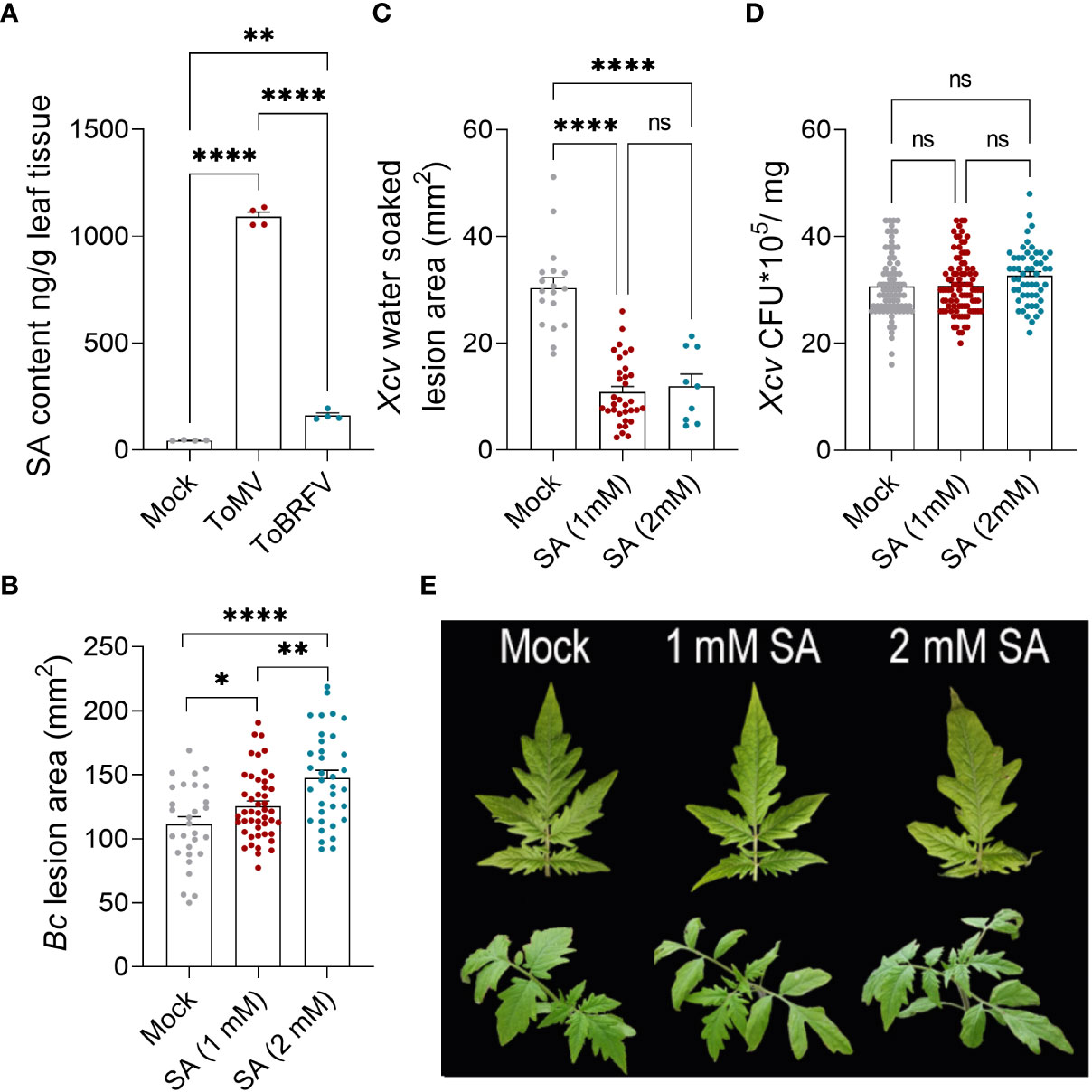
Figure 5 Tobamovirus infection alters pathogen susceptibility through the SA pathway. (A) S. lycopersicum cv. Moneymaker plants were infected with ToMV or ToBRFV at 2 weeks of age. Three weeks after viral inoculation, SA was quantified by LC-MS-MS in the fourth leaf of four individual plants. (B-D) S. lycopersicum cv. Moneymaker plants were treated with indicated concentrations of SA by foliar spray for 2 weeks (4 treatments in total). Three days after the last treatment, plants were challenged with B. cinerea 3-day-old mycelia (B) or X. euvesicatoria (Xcv, OD600 = 0.0002, (C, D) on leaves 4-5. (B) Necrotic lesion area was quantified 3 days after B. cinerea inoculation. Experiment was repeated three independent times. (C) Water-soaked lesion area was quantified 10 days after X. euvesicatoria inoculation. Experiment was repeated four independent times. (D) Bacterial CFU were quantified 5 days after X. euvesicatoria inoculation. Experiment was repeated four independent times. (E) Representative images of SA treated plants after four treatments. Bars represent mean ± SE, all points shown. Asterisks indicate statistically significant differences between indicated samples in Welch’s ANOVA with Dunnett’s post hoc test. A: N=4, p<0.005. B: N>30, p<0.036. C: N>9, p<0.0001. D: N>50, p>0.1. *p<0.05, **p<0.01, ****p<0.0001, ns, not significant.
Elicitation of SA production and alterations in disease response and cellular immunity were more pronounced with ToMV infection (Figures 2-4). Since SA levels have been shown to affect disease responses (Liu et al., 2016; Di et al., 2017; Betsuyaku et al., 2018; Li et al., 2019), we investigated whether treating tomato plants with exogenous SA at similar levels and durations elicited by 2-to-3-week tobamovirus infections could affect disease responses in a similar manner to viral infection. Tomato plants were treated with 1 mM (similar to levels measured following ToBRFV infection) or 2 mM SA. We used 2 mM to simulate ToMV infection, though it is much lower than the endogenous levels of SA elicited by ToMV, which corresponds to about 8 mM, because exogenous treatment with 5 mM SA was toxic to tomato plants (Supplementary Figure S2). SA treatments at both concentrations resulted in increased severity of gray mold disease, and moderation of X. euvesicatoria-elicited symptoms (Figures 5B, C). Bacterial load remained unaffected in all treatments (Figure 5D). These results were similar to the effect of tobamovirus infection on the disease caused by both pathogens (Figure 2).
To further explore the role of the increase in SA in alterations observed to disease susceptibility following tobamovirus infection, we examined the effect of tobamovirus infection on disease susceptibility in the SA-deficient NahG transgenic line, which lacks endogenous SA (Mehari et al., 2015; Gupta and Bar, 2020). Interestingly, while tobamovirus infection increased the B. cinerea lesion size in control plants (cv. Moneymaker), it did not have an effect in the NahG transgenic tomato line (Figures 6A, C). In addition, while ToMV infection reduced the X. euvesicatoria water soaked lesion area in control plants, it had no effect over lesion size in NahG plants (Figures 6B, C). Collectively, these results suggest that tobamovirus infection alters the plant response to B. cinerea and X. euvesicatoria in an SA-dependent manner.
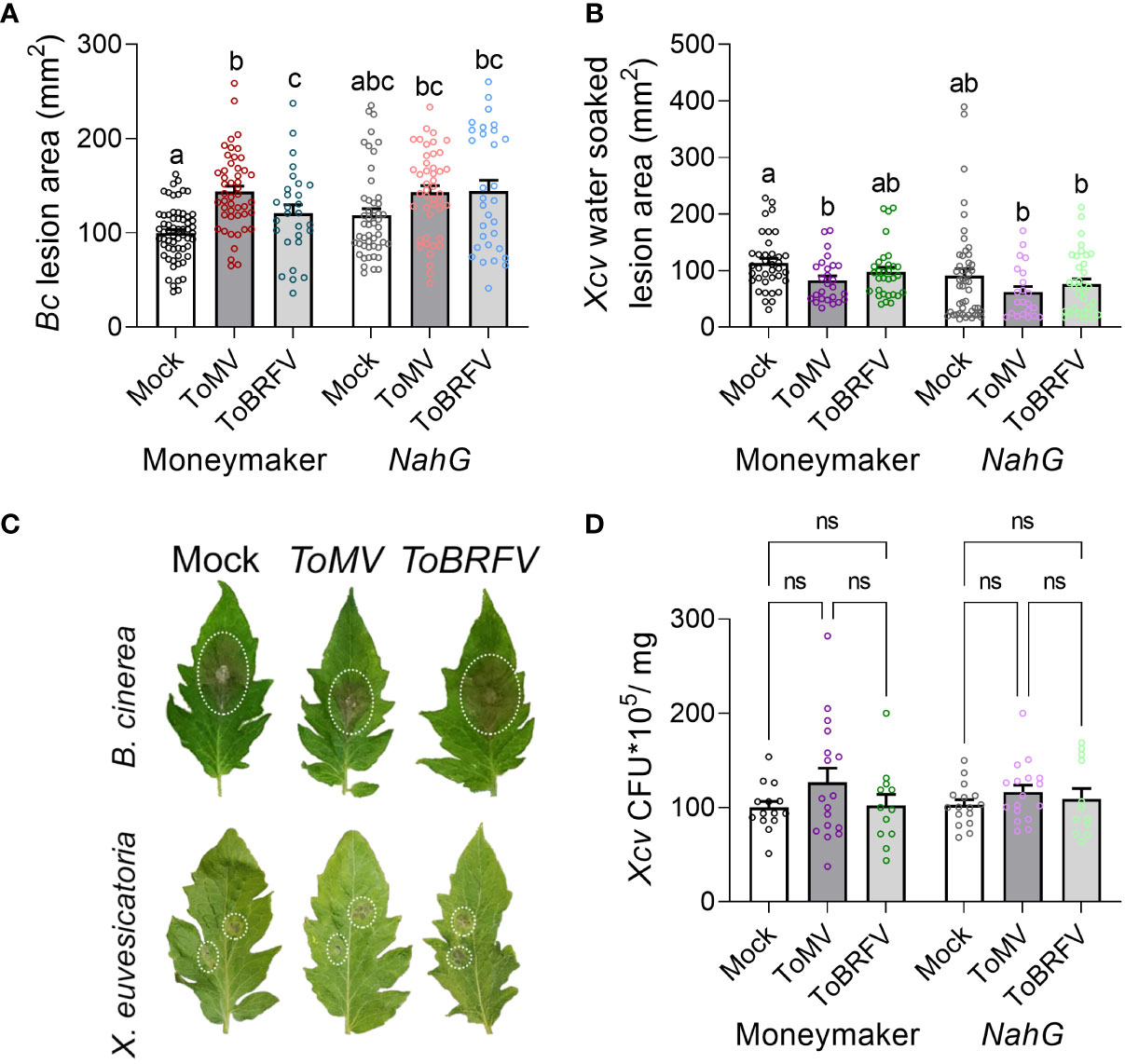
Figure 6 Tobamovirus infection requires SA to modulate pathogen resistance. S. lycopersicum cv. Moneymaker control plants or SA-degrading “NahG” transgenic plants were infected with ToMV or ToBRFV at 2 weeks of age. Three weeks after viral inoculation, plants were challenged with B. cinerea 3-day-old mycelia (A, C) or X. euvesicatoria (Xcv, OD600 = 0.0002, (B-D). (A) Necrotic lesion area was quantified 3 days after B. cinerea inoculation. Experiment was repeated 4 independent times. (B) Water-soaked lesion area, and (D) bacterial CFU, were quantified 10 and 5 days after X. euvesicatoria inoculation, respectively. Experiment was repeated 3 independent times. (C) Representative images of mock, ToMV- and ToBRFV- infected NahG plants inoculated with B. cinerea or X. euvesicatoria. Bars represent mean ± SE, all points shown. Different letters indicate statistically significant differences between samples in one way ANOVA with Tukey’s post hoc test (A), or in Welch’s ANOVA with Dunnett’s post hoc test (B, D). A: N>28, p<0.011. B: N>22, p<0.037. D: N>12, p>0.52. ns, not significant.
Discussion
Plants often encounter multiple pathogens in their environment (Abdullah et al., 2017). In addition to possible interactions between two or more pathogenic microorganisms on or within the plant, the interaction of each pathogen with the plant host can shape the hosts’ subsequent response to additional pathogens. Interactions between plant-infecting microorganisms can be symbiotic, synergistic, or antagonistic, either directly, or through the plant host (Abdullah et al., 2017). In our work, we found that the tobamoviruses ToMV and ToBRFV cause aggravation of the disease caused by the necrotrophic fungal pathogen B. cinerea, and moderation of the disease caused by the hemibiotrophic bacterial pathogen X. euvesicatoria (Figure 2). These interactions are likely generated by the response of the tomato host, through increases in SA levels (Figure 5), which aggravated the symptoms caused by the necrotroph B. cinera and attenuated the symptoms of the biotroph X. euvesicatoria. Comparison between the two studied tobamoviruses demonstrates that ToMV promoted greater increases in SA levels, stronger susceptibility to B. cinerea, and resistance to X. euvesicatoria, while ToBRFV promoted milder increases in SA levels, weaker susceptibility to B. cinerea, and no resistance to X. euvesicatoria (Figures 2, 5). Exogenous SA treatment also resulted in susceptibility to B. cinerea elicited disease and resistance to X. euvesicatoria elicited disease (Figure 5), suggesting that these differential responses observed in virus-infected plants are due to increased SA levels. In addition, NahG plants lacking endogenous SA were not able to respond to tobamovirus infection with increases in B. cinerea susceptibility or X. euvesicatoria resistance (Figure 6). Taken together, these findings support the hypothesis that the effects of viral infection on pathogen susceptibility observed in our system indeed involve SA-regulated pathways. It is important to note that when studying dual infections, the relative timing of both infections may alter the experimental outcome. In our system, the second pathogen infection occurred after systemic viral infection had occurred (21 dpi) and the virus was fully established in its host. It is possible that co-infection at earlier stages, for example during the first days of infection when the virus had not yet moved out of the infected leaf, may yield different results.
SA promotes resistance to many plant pathogens, including bacteria, fungi and viruses (Peng et al., 2021), and is required for plant immunity to (hemi)biotrophic pathogens. SA-mediated immunity has typically been described as occurring via activation of the SAR pathway (Fu and Dong, 2013; Di et al., 2017). Interestingly, early investigations of plant response to the tobamovirus TMV uncovered that SA promotes plant resistance to TMV (White, 1979). Plants which are unable to accumulate SA are highly susceptible to TMV (Gaffney et al., 1993), as SA reduces viral accumulation and cell-to-cell movement (Murphy and Carr, 2002). Tobamovirus infected plants were previously shown to accumulate high levels of SA (Malamy et al., 1990; Conti et al., 2017). We also observed hyper-accumulation of endogenous SA contents in ToMV- and ToBRFV- infected plants (Figure 5). Previously, we demonstrated that the ability of ToBRFV to break Tm-22 resistance is linked to its moderated mobility (Hak and Spiegelman, 2021; Hak et al., 2023), suggesting that ToMV spreads faster than ToBRFV in a tobamovirus-susceptible background. However, since there is currently no genetic resistance to ToBRFV in tomato, it poses a much greater agricultural challenge. Interestingly, our current work demonstrates that the reduced movement of ToBRFV (Hak and Spiegelman, 2021) correlated with a lower ability to promote increases in plant SA, as compared with ToMV (Figure 5). Thus, it would seem that systemic plant movement is correlated with the plants’ SA production, and the plant activates its SA pathways at a level commensurate with the infectivity of the virus attacking it.
Elevated SA levels are known to promote susceptibility to necrotrophic pathogens, including B. cinerea (Mengiste, 2012). Exogenous SA treatment, and endogenous SA accumulation following biotrophic pathogen infection, were both previously demonstrated to increase susceptibility to the necrotrophic fungal pathogen A. brassicicola in Arabidopsis (Spoel et al., 2007), due to inhibition of JA dependent defenses by SA. Loss of Arabidopsis thaliana BOTRYTIS-INDUCED KINASE1 (BIK1) enhanced B. cinerea susceptibility in an SA-dependent manner (Veronese et al., 2006), and B. cinerea is known to activate the SA pathway in host plants (El Oirdi et al., 2011; Rossi et al., 2011). Suppression of the tobacco mitogen-activated protein kinase phosphatase (NtMKP1) negatively regulated SA-mediated tissue necrosis and as a result enhanced resistance to B. cinerea (Oka et al., 2013). Furthermore, viral infection has been demonstrated to promote susceptibility to several necrotrophic pathogens, including B. cinerea (Omar et al., 1986; Meyer and Pataky, 2010; Philosoph et al., 2018). Our results suggest that the tobamovirus-mediated hyper-accumulation of SA may increase susceptibility to B. cinerea elicited disease in a similar manner.
While SA is considered to promote resistance against viruses, in some cases, SA fails to suppress viral infection (Murphy et al., 2020). In fact, several viruses promote SA biosynthesis and signaling during systemic infection (Krečič-Stres et al., 2005; Zhou et al., 2014; Shaw et al., 2019). It is therefore possible that while SA accumulates during viral infection, viruses suppress specific elements downstream to SA to successfully infect the plant. For example, the tobamovirus movement protein suppresses callose deposition near plasmodesmata (Guenoune-Gelbart et al., 2008), an SA- dependent process (Wang et al., 2013). The coat protein suppresses the expression of several SA-responsive genes to allow systemic transport of the virus (Conti et al., 2012; Venturuzzi et al., 2021). Other SA-mediated responses, which are not suppressed by the virus, would likely remain activated (Laird et al., 2013; Yang et al., 2016). Alternatively, the upregulation of SA could reflect a failed defense response, which is initiated after the virus is already established. This type of dysfunctional immune response may cause deleterious effects, including enhanced disease symptoms. Indeed, several studies have shown that strong viral symptoms are often associated with upregulation of the SA-responsive genes and metabolites (Diaz-Vivancos et al., 2006; Hernández et al., 2006; Niehl et al., 2006; Kogovšek et al., 2010; Shang et al., 2010). These observations suggest that during viral infection, the upregulation of several SA-mediated pathway elements may enhance the disease symptoms rather than protect plants against the virus. We propose that another effect of this immune activation may be the alteration of plant response to other pathogens.
Reportedly, SAR depends on the SA pathway, while ISR relies on JA/ET pathways. While SA is antagonistic to JA in defense signaling (Pieterse et al., 2009; Pieterse et al., 2012), there are cases where SA and JA were reported to positively interact (Ferrari et al., 2003; De Vleesschauwer et al., 2013; Liu et al., 2016; Ullah et al., 2022). Dependence of ISR on SA signaling has been reported as well (Martínez-Medina et al., 2013). Distinctions between ISR and SAR are not clear cut in tomato, and overlap between the two was reported (Liu et al., 2016; Betsuyaku et al., 2018). Thus, while plant defense against B. cinerea requires the JA-mediated pathway (AbuQamar et al., 2008), and plant defense against viruses involves the SA-mediated pathway (White, 1979; Malamy et al., 1990), we found that the plants response to tobamoviruses and B. cinerea operates in the same direction, with virus-infected plants becoming more susceptible to B. cinerea, in a SA-dependent manner (Figures 2; 5-6). This is likely due to antagonism between the high levels of SA elicited by the viral infection (Figure 5), and JA required to promote plant defenses against B. cinerea.
It should be noted that the SA-mediated effects on B. cinerea pathogenesis may also depend on the specific concentration of SA. For example, low levels of the SA analog acibenzolar-S-methyl promoted resistance to B. cinerea in tomato (Leibman-Markus et al., 2023), and priming SA-mediated defenses was also found to induce B. cinerea resistance (Audenaert et al., 2002; Gupta et al., 2020; Gupta et al., 2022; Marash et al., 2022). Here, ToMV infection resulted in SA levels of approximately 1000 ng/gr, which is equivalent to ~8mM. However, exogenous application of 5 mM resulted in phytotoxicity. Interestingly, we found that during tobamovirus infection, the levels of the SA receptor NPR1 are down-regulated (Figure 4A). This negative feedback mechanism may curb the SA response even when SA levels are exceedingly high, and may explain how the plant tolerates high endogenous doses of SA during infection. Nevertheless, the excess levels of SA may still create conditions that are favorable for necrotrophs by promoting cell death.
Our results lead to the conclusion that in certain cases, co-infection with biotrophs and necrotrophs, or SA-dependent and JA-dependent pathogens, could be particularly devastating, because the strong plant response to one type of pathogen makes the host unable to properly respond to another type of pathogen. This could have implications both in co-infection research and in agricultural cultivation. Different pathogens can be inexplicably aggressive in particular growing seasons, and it is plausible that one of the underlying causes of this might be strong activation of one pathway of the plant immune or stress response system, leaving the plant unable to respond to subsequent challenge. However, this is only relevant to a sub-set of phytopathogens and plant host systems, as viral infection was also reported to increase susceptibility to biotrophic pathogens (Latch and Potter, 1977; Bains and Parkash, 1984), and SA and JA are known to have both positive and negative interactions in plant defense (Takahashi et al., 2004; Li et al., 2019; Vlot et al., 2021; Ullah et al., 2022). These findings need to be taken into consideration when designing induced resistance protocols to mitigate plant viruses. While SAR-inducing compounds, such as acibenzolar-s-methyl were found to be effective against some viruses (Palukaitis et al., 2017), they may have a negative effect on the disease response to other pathogens, when co-infection occurs. The use of specific resistance inducers should therefore be tailored according to the needs of the specific crop, taking into account the additional potential pathogens within each specific cropping environment.
Based on our results it seems that tobamoviruses, which pose a substantial economic problem in worldwide agriculture (Zhang et al., 2022a), can also increase crop losses by making plants more susceptible to other classes of pathogens. This could be possibly mitigated by the fact that the same virus or viruses can generate some resistance to other classes of pathogens. Thus, the balance between pathogen resistance and pathogen susceptibility in a particular growing season, when dealing with tobamovirus infected plants, will depend on the prevalence of particular pathogens in the cropping environment. Our results indicate that resistance or susceptibility to different classes of pathogens in tobamovirus-infected plants are primarily a result of substantial changes to the SA pathway. Further research is needed to elucidate the relationships between different defense pathways during tobamovirus infection, as well as the roles of SA in B. cinerea pathogenesis and its interactions with the plant host.
Data availability statement
The original contributions presented in the study are included in the article/Supplementary Material. Further inquiries can be directed to the corresponding authors.
Author contributions
ZS and MB: conceptualization. ML-M, RG, DW, ZS and MB: methodology. ML-M, RG, and DW: investigation. ML-M, RG, DW, ZS and MB: analysis. ZS and MB: writing – original draft. ML-M, RG, DW, ZS and MB: writing – review and editing. ZS and MB: funding acquisition. All authors contributed to the article and approved the submitted version.
Funding
This work was partly supported by the Chief Scientist of the Israeli Ministry of Agriculture and Rural Development, Grant No. 20-02-0024, and by the Israeli Ministry of Science, Maimonides ISRAEL-FRANCE Grant No. 0002427.
Acknowledgments
We thank members of the Spiegelman and Bar groups for continuous discussion and support.
Conflict of interest
The authors declare that the research was conducted in the absence of any commercial or financial relationships that could be construed as a potential conflict of interest.
Publisher’s note
All claims expressed in this article are solely those of the authors and do not necessarily represent those of their affiliated organizations, or those of the publisher, the editors and the reviewers. Any product that may be evaluated in this article, or claim that may be made by its manufacturer, is not guaranteed or endorsed by the publisher.
Supplementary material
The Supplementary Material for this article can be found online at: https://www.frontiersin.org/articles/10.3389/fpls.2023.1196456/full#supplementary-material
Supplementary Figure 1 | Assessment of tobamovirus infection.
Supplementary Figure 2 | 5mM SA is phytotoxic.
Supplementary Table 1 | Primer pairs used in qRT-PCR.
References
Abdullah, A. S., Moffat, C. S., Lopez-Ruiz, F. J., Gibberd, M. R., Hamblin, J., Zerihun, A. (2017). Host–Multi-Pathogen warfare: pathogen interactions in Co-infected plants. Front. Plant Sci. 8. doi: 10.3389/fpls.2017.01806
AbuQamar, S., Chai, M. F., Luo, H., Song, F., Mengiste, T. (2008). Tomato protein kinase 1b mediates signaling of plant responses to necrotrophic fungi and insect herbivory. Plant Cell 20, 1964–1983. doi: 10.1105/tpc.108.059477
Aerts, N., Pereira Mendes, M., Van Wees, S. C. M. (2021). Multiple levels of crosstalk in hormone networks regulating plant defense. Plant J. 105, 489–504. doi: 10.1111/tpj.15124
Alon, D. M., Hak, H., Bornstein, M., Pines, G., Spiegelman, Z. (2021). Differential detection of the tobamoviruses tomato mosaic virus (ToMV) and tomato brown rugose fruit virus (ToBRFV) using CRISPR-Cas12a. Plants 10, 1256. doi: 10.3390/plants10061256
Anand, G., Leibman-Markus, M., Elkabetz, D., Bar, M. (2021). Method for the production and purification of plant immuno-active xylanase from trichoderma. Int. J. Mol. Sci. 22, 4214. doi: 10.3390/ijms22084214
Audenaert, K., Pattery, T., Cornelis, P., Höfte, M. (2002). Induction of systemic resistance to botrytis cinerea in tomato by pseudomonas aeruginosa 7NSK2: role of salicylic acid, pyochelin, and pyocyanin. Mol. Plant-Microbe Interact. 15, 1147–1156. doi: 10.1094/MPMI.2002.15.11.1147
Bains, S. S., Parkash, V. (1984). Increased susceptibility toPseudoperonospora cubensis in virus-infectedCucurbita pepo genotypes. Phytoparasitica 12, 183–188. doi: 10.1007/BF02981171
Betsuyaku, S., Katou, S., Takebayashi, Y., Sakakibara, H., Nomura, N., Fukuda, H. (2018). Salicylic acid and jasmonic acid pathways are activated in spatially different domains around the infection site during effector-triggered immunity in arabidopsis thaliana. Plant Cell Physiol. 59, 8–16. doi: 10.1093/pcp/pcx181
Broadbent, L. (1976). Epidemiology and control of tomato mosaic virus. Annu. Rev. Phytopathol. 14, 75–96. doi: 10.1146/annurev.py.14.090176.000451
Caarls, L., Pieterse, C. M. J., Van Wees, S. C. M. (2015). How salicylic acid takes transcriptional control over jasmonic acid signaling. Front. Plant Sci. 6. doi: 10.3389/fpls.2015.00170
Cao, H., Glazebrook, J., Clarke, J. D., Volko, S., Dong, X. (1997). The arabidopsis NPR1 gene that controls systemic acquired resistance encodes a novel protein containing ankyrin repeats. Cell 88, 57–63. doi: 10.1016/s0092-8674(00)81858-9
Conti, G., Rodriguez, M. C., Manacorda, C. A., Asurmendi, S. (2012). Transgenic expression of tobacco mosaic virus capsid and movement proteins modulate plant basal defense and biotic stress responses in nicotiana tabacum. MPMI 25, 1370–1384. doi: 10.1094/MPMI-03-12-0075-R
Conti, G., Rodriguez, M. C., Venturuzzi, A. L., Asurmendi, S. (2017). Modulation of host plant immunity by tobamovirus proteins. Ann. Bot. 119, 737–747. doi: 10.1093/aob/mcw216
Cooper, A. J., Latunde-Dada, A. O., Woods-Tör, A., Lynn, J., Lucas, J. A., Crute, I. R., et al. (2008). Basic compatibility of albugo candida in arabidopsis thaliana and brassica juncea causes broad-spectrum suppression of innate immunity. MPMI 21, 745–756. doi: 10.1094/MPMI-21-6-0745
Davino, S., Caruso, A. G., Bertacca, S., Barone, S., Panno, S. (2020). Tomato brown rugose fruit virus: seed transmission rate and efficacy of different seed disinfection treatments. Plants 9, 1615. doi: 10.3390/plants9111615
Delplace, F., Huard-Chauveau, C., Dubiella, U., Khafif, M., Alvarez, E., Langin, G., et al. (2020). Robustness of plant quantitative disease resistance is provided by a decentralized immune network. Proc. Natl. Acad. Sci. United States America 117, 18099–18109. doi: 10.1073/pnas.2000078117
De Vleesschauwer, D., Gheysen, G., Höfte, M. (2013). Hormone defense networking in rice: tales from a different world. Trends Plant Sci. 18, 555–565. doi: 10.1016/j.tplants.2013.07.002
D’haene, B., Vandesompele, J., Hellemans, J. (2010). Accurate and objective copy number profiling using real-time quantitative PCR. Methods 50, 262–270. doi: 10.1016/j.ymeth.2009.12.007
Di, X., Gomila, J., Takken, F. L. W. (2017). Involvement of salicylic acid, ethylene and jasmonic acid signalling pathways in the susceptibility of tomato to Fusarium oxysporum. Mol. Plant Pathol. 18, 1024–1035. doi: 10.1111/mpp.12559
Diaz-Vivancos, P., Rubio, M., Mesonero, V., Periago, P., Ros Barceló, A., Martínez-Gómez, P., et al. (2006). The apoplastic antioxidant system in prunus: response to long-term plum pox virus infection. J. Exp. Bot. 57, 3813–3824. doi: 10.1093/jxb/erl138
Dombrovsky, A., Mor, N., Gantz, S., Lachman, O., Smith, E. (2022). Disinfection efficacy of tobamovirus-contaminated soil in greenhouse-grown crops. Horticulturae 8, 563. doi: 10.3390/horticulturae8070563
Elad, Y., Pertot, I., Cotes Prado, A. M., Stewart, A. (2016). “Plant hosts of botrytis spp,” in Botrytis – the fungus, the pathogen and its management in agricultural systems (Cham: Springer International Publishing), 413–486. doi: 10.1007/978-3-319-23371-0_20
El Oirdi, M., El Rahman, T. A., Rigano, L., El Hadrami, A., Rodriguez, M. C., Daayf, F., et al. (2011). Botrytis cinerea manipulates the antagonistic effects between immune pathways to promote disease development in tomato. Plant Cell 23, 2405–2421. doi: 10.1105/tpc.111.083394
Fang, K., Zhou, J., Chen, L., Li, Y.-X., Yang, A.-L., Dong, X.-F., et al. (2021). Virulence and community dynamics of fungal species with vertical and horizontal transmission on a plant with multiple infections. PloS Pathog. 17, e1009769. doi: 10.1371/journal.ppat.1009769
Ferrari, S., Plotnikova, J. M., De Lorenzo, G., Ausubel, F. M. (2003). Arabidopsis local resistance to botrytis cinerea involves salicylic acid and camalexin and requires EDS4 and PAD2, but not SID2, EDS5 or PAD4. Plant J. 35, 193–205. doi: 10.1046/j.1365-313X.2003.01794.x
Fillinger, S., Elad, Y. (2016) Botrytis – the fungus, the pathogen and its management in agricultural systems. Available at: https://books.google.co.il/books/about/Botrytis_the_Fungus_the_Pathogen_and_Its.html?id=1FsNswEACAAJ&redir_esc=y (Accessed February 27, 2018).
Fu, Z. Q., Dong, X. (2013). Systemic acquired resistance: turning local infection into global defense. Annu. Rev. Plant Biol. 64, 839–863. doi: 10.1146/annurev-arplant-042811-105606
Gaffney, T., Friedrich, L., Vernooij, B., Negrotto, D., Nye, G., Uknes, S., et al. (1993). Requirement of salicylic acid for the induction of systemic acquired resistance. Science 261, 754–756. doi: 10.1126/science.261.5122.754
Gu, Y.-Q., Wildermuth, M. C., Chakravarthy, S., Loh, Y.-T., Yang, C., He, X., et al. (2002). Tomato transcription factors pti4, pti5, and pti6 activate defense responses when expressed in arabidopsis. Plant Cell 14, 817–831. doi: 10.1105/tpc.000794
Guenoune-Gelbart, D., Elbaum, M., Sagi, G., Levy, A., Epel, B. L. (2008). Tobacco mosaic virus (TMV) replicase and movement protein function synergistically in facilitating TMV spread by lateral diffusion in the plasmodesmal desmotubule of nicotiana benthamiana. MPMI 21, 335–345. doi: 10.1094/MPMI-21-3-0335
Gupta, R., Bar, M. (2020). “Plant immunity, priming, and systemic resistance as mechanisms for trichoderma spp. biocontrol,” Trichoderma: Host Pathogen Interactions and Applications (Singapore: Springer), 81–110. doi: 10.1007/978-981-15-3321-1_5
Gupta, R., Leibman-Markus, M., Anand, G., Rav David, D., Yermiyahu, U., Elad, Y., et al. (2022). Nutrient elements promote disease resistance in tomato by differentially activating immune pathways. Phytopathology. 112 (11), 2360–2370. doi: 10.1094/PHYTO-02-22-0052-R
Gupta, R., Leibman-Markus, M., Marash, I., Kovetz, N., Rav-David, D., Elad, Y., et al. (2021a). Root zone warming represses foliar diseases in tomato by inducing systemic immunity. Plant Cell Environ. 44, 2277–2289. doi: 10.1111/pce.14006
Gupta, R., Pizarro, L., Leibman-Markus, M., Marash, I., Bar, M. (2020). Cytokinin response induces immunity and fungal pathogen resistance, and modulates trafficking of the PRR LeEIX2 in tomato. Mol. Plant Pathol. 21, 10.1111/mpp.12978. doi: 10.1111/mpp.12978
Gupta, R., Leibman-Markus, M., Pizarro, L., Bar, M. (2021b). Cytokinin induces bacterial pathogen resistance in tomato. Plant Pathol. 70, 318–325. doi: 10.1111/ppa.13279
Hak, H., Raanan, H., Schwarz, S., Sherman, Y., Dinesh-Kumar, S. P., Spiegelman, Z. (2023). Activation of Tm-22 resistance is mediated by a conserved cysteine essential for tobacco mosaic virus movement. Mol. Plant Pathol. doi: 10.1111/mpp.13318
Hak, H., Spiegelman, Z. (2021). The tomato brown rugose fruit virus movement protein overcomes Tm-22 resistance in tomato while attenuating viral transport. Mol. Plant Microbe Interact. 34, 1024–1032. doi: 10.1094/MPMI-01-21-0023-R
Hall, T. J. (1980). Resistance at the TM-2 locus in the tomato to tomato mosaic virus. Euphytica 29, 189–197. doi: 10.1007/BF00037266
Hernández, J. A., Díaz-Vivancos, P., Rubio, M., Olmos, E., Ros-Barceló, A., Martínez-Gómez, P. (2006). Long-term plum pox virus infection produces an oxidative stress in a susceptible apricot, prunus armeniaca, cultivar but not in a resistant cultivar. Physiologia Plantarum 126, 140–152. doi: 10.1111/j.1399-3054.2005.00581.x
Ishiga, Y., Ishiga, T., Uppalapati, S. R., Mysore, K. S. (2013). Jasmonate ZIM-domain (JAZ) protein regulates host and nonhost pathogen-induced cell death in tomato and nicotiana benthamiana. PloS One 8, e75728. doi: 10.1371/journal.pone.0075728
Jones, J. D. G., Dangl, J. L. (2006). The plant immune system. Nature 444, 323–329. doi: 10.1038/nature05286
Jones, J. B., Lacy, G. H., Bouzar, H., Stall, R. E., Schaad, N. W. (2004). Reclassification of the xanthomonads associated with bacterial spot disease of tomato and pepper. Systematic Appl. Microbiol. 27, 755–762. doi: 10.1078/0723202042369884
Kim, D. S., Hwang, B. K. (2014). An important role of the pepper phenylalanine ammonia-lyase gene (PAL1) in salicylic acid-dependent signalling of the defence response to microbial pathogens. J. Exp. Bot. 65, 2295–2306. doi: 10.1093/jxb/eru109
Klessig, D. F., Choi, H. W., Dempsey, D. A. (2018). Systemic acquired resistance and salicylic acid: past, present, and future. Mol. Plant-Microbe Interact. 31, 871–888. doi: 10.1094/MPMI-03-18-0067-CR
Kogovšek, P., Pompe-Novak, M., Baebler, Š., Rotter, A., Gow, L., Gruden, K., et al. (2010). Aggressive and mild potato virus y isolates trigger different specific responses in susceptible potato plants. Plant Pathol. 59, 1121–1132. doi: 10.1111/j.1365-3059.2010.02340.x
Kravchik, M., Shnaider, Y., Abebie, B., Shtarkman, M., Kumari, R., Kumar, S., et al. (2022). Knockout of SlTOM1 and SlTOM3 results in differential resistance to tobamovirus in tomato. Mol. Plant Pathol. 23, 1278–1289. doi: 10.1111/mpp.13227
Krečič-Stres, H., Vučak, C., Ravnikar, M., Kovač, M. (2005). Systemic potato virus YNTN infection and levels of salicylic and gentisic acids in different potato genotypes. Plant Pathol. 54, 441–447. doi: 10.1111/j.1365-3059.2005.01209.x
Laird, J., McInally, C., Carr, C., Doddiah, S., Yates, G., Chrysanthou, E., et al. (2013). Identification of the domains of cauliflower mosaic virus protein P6 responsible for suppression of RNA silencing and salicylic acid signalling. J. Gen. Virol. 94, 2777–2789. doi: 10.1099/vir.0.057729-0
Lamichhane, J. R., Venturi, V. (2015). Synergisms between microbial pathogens in plant disease complexes: a growing trend. Front. Plant Sci. 6. doi: 10.3389/fpls.2015.00385
Latch, G. C. M., Potter, L. R. (1977). Interaction between crown rust (Puccinia coronata) and two viruses of ryegrass. Ann. Appl. Biol. 87, 139–145. doi: 10.1111/j.1744-7348.1977.tb01869.x
Leibman-Markus, M., Schuster, S., Avni, A. (2017). “LeEIX2 interactors’ analysis and EIX-mediated responses measurement,” in Plant pattern recognition receptors: methods and protocols. Eds. Shan, L., He, P. (New York, NY: Springer New York), 167–172. doi: 10.1007/978-1-4939-6859-6_13
Leibman-Markus, M., Schneider, A., Gupta, R., Marash, I., Rav-David, D., Weissberg-Carmeli, M., et al. (2023). Immunity priming uncouples the growth-defense tradeoff in tomato. bioRxiv. doi: 10.1101/2022.07.24.501304
Li, N., Han, X., Feng, D., Yuan, D., Huang, L.-J. (2019). Signaling crosstalk between salicylic acid and Ethylene/Jasmonate in plant defense: do we understand what they are whispering? Int. J. Mol. Sci. 20 (3), 671. doi: 10.3390/ijms20030671
Lin, W.-C., Lu, C.-F., Wu, J.-W., Cheng, M.-L., Lin, Y.-M., Yang, N.-S., et al. (2004). Transgenic tomato plants expressing the arabidopsis NPR1 gene display enhanced resistance to a spectrum of fungal and bacterial diseases. Transgenic Res. 13, 567–581. doi: 10.1007/s11248-004-2375-9
Liu, L., Sonbol, F.-M., Huot, B., Gu, Y., Withers, J., Mwimba, M., et al. (2016). Salicylic acid receptors activate jasmonic acid signalling through a non-canonical pathway to promote effector-triggered immunity. Nat. Commun. 7 (1), 13099. doi: 10.1038/NCOMMS13099
Lund, S. T., Stall, R. E., Klee, H. J. (1998). Ethylene regulates the susceptible response to pathogen infection in tomato. Plant Cell 10, 371–382. doi: 10.1105/tpc.10.3.371
Luria, N., Smith, E., Reingold, V., Bekelman, I., Lapidot, M., Levin, I., et al. (2017). A new Israeli tobamovirus isolate infects tomato plants harboring Tm-22 resistance genes. PloS One 12, e0170429. doi: 10.1371/journal.pone.0170429
Malamy, J., Carr, J. P., Klessig, D. F., Raskin, I. (1990). Salicylic acid: a likely endogenous signal in the resistance response of tobacco to viral infection. Science 250, 1002–1004. doi: 10.1126/science.250.4983.1002
Marash, I., Leibman-Markus, M., Gupta, R., Avni, A., Bar, M. (2022). TOR inhibition primes immunity and pathogen resistance in tomato in a salicylic acid-dependent manner. Mol. Plant Pathol. 23, 1035–1047. doi: 10.1111/mpp.13207
Martínez-Medina, A., Fernández, I., Sánchez-Guzmán, M. J., Jung, S. C., Pascual, J. A., Pozo, M. J. (2013). Deciphering the hormonal signalling network behind the systemic resistance induced by trichoderma harzianum in tomato. Front. Plant Sci. 4. doi: 10.3389/fpls.2013.00206
Mascia, T., Santovito, E., Gallitelli, D., Cillo, F. (2010). Evaluation of reference genes for quantitative reverse-transcription polymerase chain reaction normalization in infected tomato plants. Mol. Plant Pathol. 11, 805–816. doi: 10.1111/j.1364-3703.2010.00646.x
Mehari, Z. H., Elad, Y., Rav-David, D., Graber, E. R., Meller Harel, Y. (2015). Induced systemic resistance in tomato (Solanum lycopersicum) against botrytis cinerea by biochar amendment involves jasmonic acid signaling. Plant Soil 395, 31–44. doi: 10.1007/s11104-015-2445-1
Meller Harel, Y., Mehari, Z. H., Rav-David, D., Elad, Y. (2014). Systemic resistance to Gray mold induced in tomato by benzothiadiazole and Trichoderma harzianum T39. Phytopathology 104, 150–157. doi: 10.1094/PHYTO-02-13-0043-R
Mengiste, T. (2012). Plant immunity to necrotrophs. Annu. Rev. Phytopathol. 50, 267–294. doi: 10.1146/annurev-phyto-081211-172955
Meyer, M. D., Pataky, J. K. (2010). Increased severity of foliar diseases of sweet corn infected with maize dwarf mosaic and sugarcane mosaic viruses. Plant Dis. 94, 1093–1099. doi: 10.1094/PDIS-94-9-1093
Moss, W. P., Byrne, J. M., Campbell, H. L., Ji, P., Bonas, U., Jones, J. B., et al. (2007). Biological control of bacterial spot of tomato using hrp mutants of xanthomonas campestris pv. vesicatoria. Biol. Control 41, 199–206. doi: 10.1016/J.BIOCONTROL.2007.01.008
Murphy, A. M., Carr, J. P. (2002). Salicylic acid has cell-specific effects on tobacco mosaic virus replication and cell-to-cell movement. Plant Physiol. 128, 552–563. doi: 10.1104/pp.010688
Murphy, A. M., Zhou, T., Carr, J. P. (2020). An update on salicylic acid biosynthesis, its induction and potential exploitation by plant viruses. Curr. Opin. Virol. 42, 8–17. doi: 10.1016/j.coviro.2020.02.008
Niehl, A., Lacomme, C., Erban, A., Kopka, J., Krämer, U., Fisahn, J., et al. (2006). Systemic potato virus X infection induces defence gene expression and accumulation of β-phenylethylamine-alkaloids in potato. Funct. Plant Biol. 33, 593–604. doi: 10.1071/FP06049
O’Donnell, P. J., Jones, J. B., Antoine, F. R., Ciardi, J., Klee, H. J. (2001). Ethylene-dependent salicylic acid regulates an expanded cell death response to a plant pathogen. Plant J. 25, 315–323. doi: 10.1046/j.1365-313x.2001.00968.x
Oka, K., Amano, Y., Katou, S., Seo, S., Kawazu, K., Mochizuki, A., et al. (2013). Tobacco MAP kinase phosphatase (NtMKP1) negatively regulates wound response and induced resistance against necrotrophic pathogens and lepidopteran herbivores. MPMI 26, 668–675. doi: 10.1094/MPMI-11-12-0272-R
Oladokun, J. O., Halabi, M. H., Barua, P., Nath, P. D. (2019). Tomato brown rugose fruit disease: current distribution, knowledge and future prospects. Plant Pathol. 68, 1579–1586. doi: 10.1111/ppa.13096
Omar, S. A. M., Bailiss, K. W., Chapman, G. P. (1986). Virus-induced changes in the response of faba bean to infection by botrytis. Plant Pathol. 35, 86–92. doi: 10.1111/j.1365-3059.1986.tb01985.x
Orton, E. S., Brown, J. K. M. (2016). Reduction of growth and reproduction of the biotrophic fungus blumeria graminis in the presence of a necrotrophic pathogen. Front. Plant Sci. 7. doi: 10.3389/fpls.2016.00742
Palukaitis, P., Yoon, J.-Y., Choi, S.-K., Carr, J. P. (2017). Manipulation of induced resistance to viruses. Curr. Opin. Virol. 26, 141–148. doi: 10.1016/j.coviro.2017.08.001
Panno, S., Ruiz-Ruiz, S., Caruso, A. G., Alfaro-Fernandez, A., Ambrosio, M. I. F. S., Davino, S. (2019). Real-time reverse transcription polymerase chain reaction development for rapid detection of tomato brown rugose fruit virus and comparison with other techniques. PeerJ 7, e7928. doi: 10.7717/peerj.7928
Peng, Y., Yang, J., Li, X., Zhang, Y. (2021). Salicylic acid: biosynthesis and signaling. Annu. Rev. Plant Biol. 72, 761–791. doi: 10.1146/annurev-arplant-081320-092855
Philosoph, A. M., Dombrovsky, A., Elad, Y., Jaiswal, A. K., Koren, A., Lachman, O., et al. (2018). Combined infection with Cucumber green mottle mosaic virus and Pythium species causes extensive collapse in cucumber plants. Plant Dis. 102, 753–759. doi: 10.1094/PDIS-07-17-1124-RE
Philosoph, A. M., Dombrovsky, A., Elad, Y., Koren, A., Frenkel, O. (2019). Insight into late wilting disease of cucumber demonstrates the complexity of the phenomenon in fluctuating environments. Plant Dis. 103, 2877–2883. doi: 10.1094/PDIS-12-18-2141-RE
Pieterse, C. M. J. J., Leon-Reyes, A., van der Ent, S., Van Wees, S. C. M. M. (2009). Networking by small-molecule hormones in plant immunity. Nature. 5 (5), 308–316. doi: 10.1038/nchembio.164
Pieterse, C. M. J., van der Does, D., Zamioudis, C., Leon-Reyes, A., Van Wees, S. C. M. (2012). Hormonal modulation of plant immunity. Annu. Rev. Cell Dev. Biol. 28, 489–521. doi: 10.1146/annurev-cellbio-092910-154055
Pizarro, L., Leibman-Markus, M., Gupta, R., Kovetz, N., Shtein, I., Bar, E., et al. (2020). A gain of function mutation in SlNRC4a enhances basal immunity resulting in broad-spectrum disease resistance. Commun. Biol. 3, 1–14. doi: 10.1038/s42003-020-01130-w
Rossi, F. R., Gárriz, A., Marina, M., Romero, F. M., Gonzalez, M. E., Collado, I. G., et al. (2011). The sesquiterpene botrydial produced by botrytis cinerea induces the hypersensitive response on plant tissues and its action is modulated by salicylic acid and jasmonic acid signaling. MPMI 24, 888–896. doi: 10.1094/MPMI-10-10-0248
Rowe, H. C., Walley, J. W., Corwin, J., Chan, E. K.-F., Dehesh, K., Kliebenstein, D. J. (2010). Deficiencies in jasmonate-mediated plant defense reveal quantitative variation in botrytis cinerea pathogenesis. PloS Pathog. 6, e1000861. doi: 10.1371/journal.ppat.1000861
Samarah, N., Sulaiman, A., Salem, N. M., Turina, M. (2021). Disinfection treatments eliminated tomato brown rugose fruit virus in tomato seeds. Eur. J. Plant Pathol. 159, 153–162. doi: 10.1007/s10658-020-02151-1
Shang, J., Xi, D.-H., Yuan, S., Xu, F., Xu, M.-Y., Qi, H.-L., et al. (2010). Difference of physiological characters in dark green islands and yellow leaf tissue of cucumber mosaic virus (CMV)-infected nicotiana tabacum leaves. Z. für Naturforschung C 65, 73–78. doi: 10.1515/znc-2010-1-213
Shaw, J., Yu, C., Makhotenko, A. V., Makarova, S. S., Love, A. J., Kalinina, N. O., et al. (2019). Interaction of a plant virus protein with the signature cajal body protein coilin facilitates salicylic acid-mediated plant defence responses. New Phytol. 224, 439–453. doi: 10.1111/nph.15994
Shaya, F., David, I., Yitzhak, Y., Izhaki, A. (2019). Hormonal interactions during early physiological partenocarpic fruitlet abscission in persimmon (Diospyros kaki thunb.) ‘Triumph’ and ‘Shinshu’ cultivars. Scientia Hortic. 243, 575–582. doi: 10.1016/j.scienta.2018.08.044
Spoel, S. H., Johnson, J. S., Dong, X. (2007). Regulation of tradeoffs between plant defenses against pathogens with different lifestyles. Proc. Natl. Acad. Sci. 104, 18842–18847. doi: 10.1073/pnas.0708139104
Takahashi, H., Kanayama, Y., Zheng, M. S., Kusano, T., Hase, S., Ikegami, M., et al. (2004). Antagonistic interactions between the SA and JA signaling pathways in arabidopsis modulate expression of defense genes and gene-for-Gene resistance to cucumber mosaic virus. Plant Cell Physiol. 45, 803–809. doi: 10.1093/pcp/pch085
Tan, J., De Zutter, N., De Saeger, S., De Boevre, M., Tran, T. M., van der Lee, T., et al. (2021). Presence of the weakly pathogenic fusarium poae in the fusarium head blight disease complex hampers biocontrol and chemical control of the virulent fusarium graminearum pathogen. Front. Plant Sci. 12. doi: 10.3389/fpls.2021.641890
Teper, D., Girija, A. M., Bosis, E., Popov, G., Savidor, A., Sessa, G. (2018). The xanthomonas euvesicatoria type III effector XopAU is an active protein kinase that manipulates plant MAP kinase signaling. PloS Pathog. 14, e1006880. doi: 10.1371/journal.ppat.1006880
Thaler, J. S., Humphrey, P. T., Whiteman, N. K. (2012). Evolution of jasmonate and salicylate signal crosstalk. Trends Plant Sci. 17, 260–270. doi: 10.1016/j.tplants.2012.02.010
Tollenaere, C., Lacombe, S., Wonni, I., Barro, M., Ndougonna, C., Gnacko, F., et al. (2017). Virus-bacteria rice Co-infection in Africa: field estimation, reciprocal effects, molecular mechanisms, and evolutionary implications. Front. Plant Sci. 8. doi: 10.3389/fpls.2017.00645
Ullah, C., Schmidt, A., Reichelt, M., Tsai, C.-J., Gershenzon, J. (2022). Lack of antagonism between salicylic acid and jasmonate signalling pathways in poplar. New Phytol. 235, 701–717. doi: 10.1111/nph.18148
Vaisman, M., Hak, H., Arazi, T., Spiegelman, Z. (2022). The impact of tobamovirus infection on root development involves induction of auxin response factor 10a in tomato. Plant Cell Physiol. 63, 1980–1993. doi: 10.1093/pcp/pcab179
Varughese, J., Griffiths, E. (1983). Effect of barley yellow dwarf virus on susceptibility of barley cultivars to net blotch (Pyrenophora teres) and leaf blotch (Rhynchosporium secalis). Plant Pathol. 32, 435–440. doi: 10.1111/j.1365-3059.1983.tb02858.x
Venturuzzi, A. L., Rodriguez, M. C., Conti, G., Leone, M., Caro, M. D. P., Montecchia, J. F., et al. (2021). Negative modulation of SA signaling components by the capsid protein of tobacco mosaic virus is required for viral long-distance movement. Plant J. 106, 896–912. doi: 10.1111/tpj.15268
Veronese, P., Nakagami, H., Bluhm, B., AbuQamar, S., Chen, X., Salmeron, J., et al. (2006). The membrane-anchored BOTRYTIS-INDUCED KINASE1 plays distinct roles in arabidopsis resistance to necrotrophic and biotrophic pathogens. Plant Cell 18, 257–273. doi: 10.1105/tpc.105.035576
Vlot, A. C., Sales, J. H., Lenk, M., Bauer, K., Brambilla, A., Sommer, A., et al. (2021). Systemic propagation of immunity in plants. New Phytol. 229, 1234–1250. doi: 10.1111/nph.16953
Walters, D., Heil, M. (2007). Costs and trade-offs associated with induced resistance. Physiol. Mol. Plant Pathol. 71, 3–17. doi: 10.1016/j.pmpp.2007.09.008
Wang, X., Sager, R., Cui, W., Zhang, C., Lu, H., Lee, J.-Y. (2013). Salicylic acid regulates plasmodesmata closure during innate immune responses in arabidopsis. Plant Cell 25, 2315–2329. doi: 10.1105/tpc.113.110676
White, R. F. (1979). Acetylsalicylic acid (aspirin) induces resistance to tobacco mosaic virus in tobacco. Virology 99, 410–412. doi: 10.1016/0042-6822(79)90019-9
Williamson, B., Tudzynski, B., Tudzynski, P., Van Kan, J. A. L. (2007). Botrytis cinerea: the cause of grey mould disease. Mol. Plant Pathol. 8, 561–580. doi: 10.1111/j.1364-3703.2007.00417.x
Yalpani, N., Silverman, P., Wilson, T. M., Kleier, D. A., Raskin, I. (1991). Salicylic acid is a systemic signal and an inducer of pathogenesis-related proteins in virus-infected tobacco. Plant Cell 3, 809–818. doi: 10.1105/tpc.3.8.809
Yang, L., Li, B., Zheng, X., Li, J., Yang, M., Dong, X., et al. (2015). Salicylic acid biosynthesis is enhanced and contributes to increased biotrophic pathogen resistance in arabidopsis hybrids. Nat. Commun. 6, 7309. doi: 10.1038/ncomms8309
Yang, L., Xu, Y., Liu, Y., Meng, D., Jin, T., Zhou, X. (2016). HC-pro viral suppressor from tobacco vein banding mosaic virus interferes with DNA methylation and activates the salicylic acid pathway. Virology 497, 244–250. doi: 10.1016/j.virol.2016.07.024
Zhang, Y., Chen, C., Mai, Z., Lin, J., Nie, L., Maharachchikumbura, S. S. N., et al. (2022b). Co-Infection of fusarium aglaonematis sp. nov. and fusarium elaeidis causing stem rot in aglaonema modestum in China. Front. Microbiol. 13. doi: 10.3389/fmicb.2022.930790
Zhang, S., Griffiths, J. S., Marchand, G., Bernards, M. A., Wang, A. (2022a). Tomato brown rugose fruit virus: an emerging and rapidly spreading plant RNA virus that threatens tomato production worldwide. Mol. Plant Pathol. 23, 1262–1277. doi: 10.1111/mpp.13229
Zhou, T., Murphy, A. M., Lewsey, M. G., Westwood, J. H., Zhang, H.-M., González, I., et al. (2014). Domains of the cucumber mosaic virus 2b silencing suppressor protein affecting inhibition of salicylic acid-induced resistance and priming of salicylic acid accumulation during infection. J. Gen. Virol. 95, 1408–1413. doi: 10.1099/vir.0.063461-0
Keywords: Botrytis cinerea, tobamovirus, ToMV, ToBRFV, salicylic acid, immunity
Citation: Gupta R, Leibman-Markus M, Weiss D, Spiegelman Z and Bar M (2023) Tobamovirus infection aggravates gray mold disease caused by Botrytis cinerea by manipulating the salicylic acid pathway in tomato. Front. Plant Sci. 14:1196456. doi: 10.3389/fpls.2023.1196456
Received: 29 March 2023; Accepted: 22 May 2023;
Published: 12 June 2023.
Edited by:
Dirk Albert Balmer, Invaio Sciences, SwitzerlandReviewed by:
Lóránt Király, Hungarian Academy of Sciences, HungaryAleksandra Obrępalska–Stęplowska, Institute of Plant Protection – National Research Institute, Poland
Copyright © 2023 Gupta, Leibman-Markus, Weiss, Spiegelman and Bar. This is an open-access article distributed under the terms of the Creative Commons Attribution License (CC BY). The use, distribution or reproduction in other forums is permitted, provided the original author(s) and the copyright owner(s) are credited and that the original publication in this journal is cited, in accordance with accepted academic practice. No use, distribution or reproduction is permitted which does not comply with these terms.
*Correspondence: Ziv Spiegelman, ziv.spi@volcani.agri.gov.il; Maya Bar, mayabar@volcani.agri.gov.il
†These authors have contributed equally to this work